What is a Superconductor?
A deep dive into the science behind superconductors.
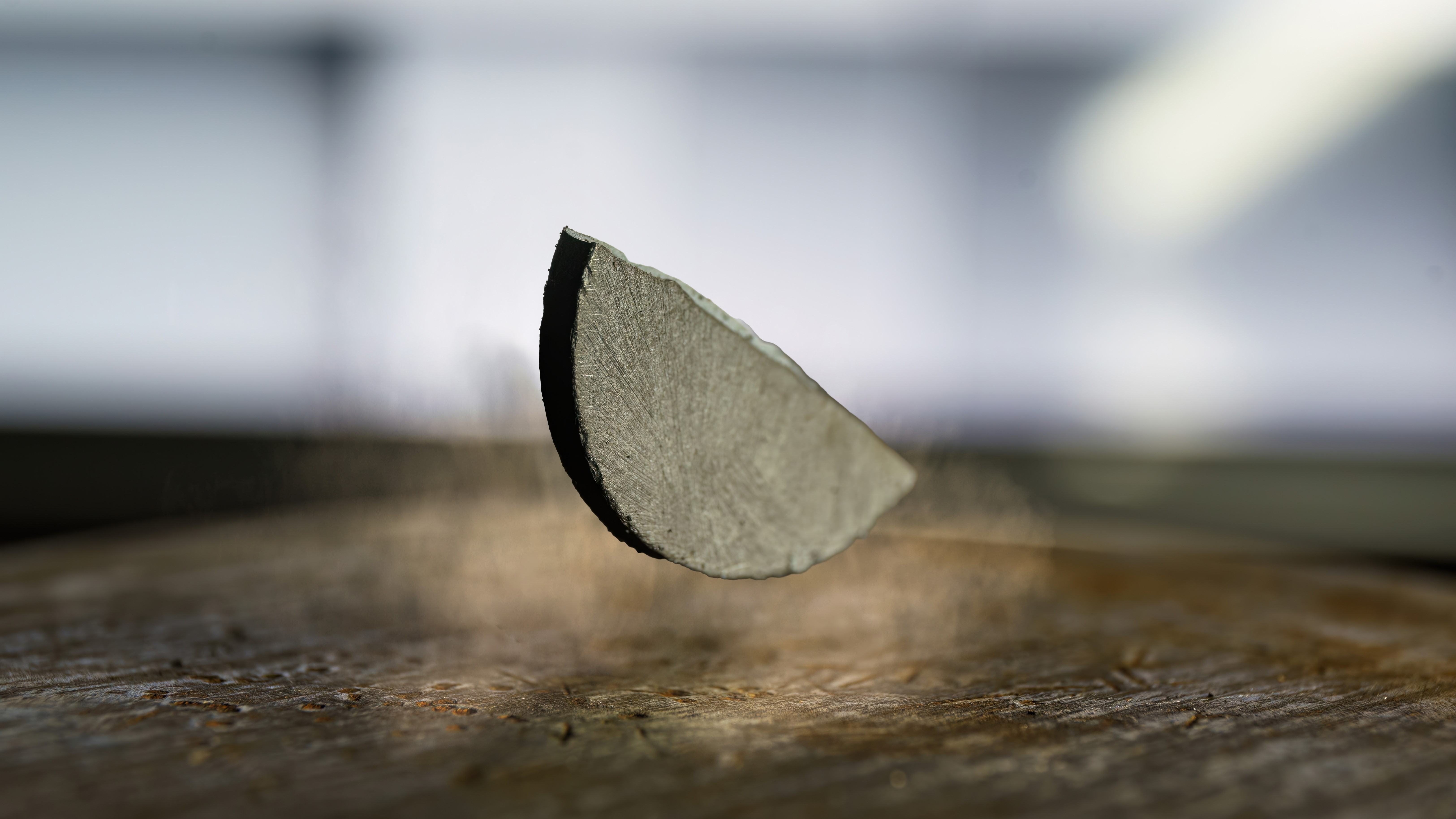
Every electric product that you use -- even the power lines bringing the electricity into the building -- suffers from energy loss as the conductive circuits and transistors are inefficient. Not only is this a waste of resources, but also the lost energy generates heat that can cause its own problems (think about your CPU and all the cooling it needs). If only there were a way to stop this waste!
Superconductors are materials that, at extremely cold temperatures, can conduct electricity at 100 percent efficiency. Should humanity be able to fabricate reliable room-temperature, ambient-pressure superconductors, many of our technological efforts would become supercharged. Our computers would become faster and operate at much lower temperatures; our powerlines could be made to work at full efficiency, with not a single watt being lost in transit; and magnetic batteries could be made that would never lose the power stored within them to entropy.
These things would be possible (and up to a point, they already are possible) because superconductors are materials with the incredible property of allowing electricity to flow without any losses to electrical resistance. Not only that, but they can also be used to build permanent magnets, opening up the door to a number of tools and inventions (such as levitating maglev trains).
But what does all this mean? What are superconductors exactly? Here’s a brief view into what superconductors are; how they work; the story and research behind them; and what our superconducting future could look like.
The Science of Superconduction
Zero Electrical Resistance
The first superconductor was created by Dutch physicist Heike Kamerlingh Onnes, who in 1911, created the first lossless “battery”. Onnes was studying the electrical properties of mercury (the same substance that expands and contracts within thermometers) when he found that mercury’s electrical resistance disappeared at temperatures below 4.2 Kelvin (4.2 degrees Celsius).
A number of other materials have been found to become superconductors – but all of them need to be cooled below a certain level. At a threshold which varies from material to material, electrical resistance abruptly drops. But to understand why this happens, we have to look at the quantum level and the Standard Model of Physics.
The world of elementary particles (the mass of particles scientists insist we’re made of) is divided in various groups. One of these elementary particles, the electron, is part of the fermionic family (this Fermi bit will be important later). And electrical current (at its most fundamental) is simply the ordered movement of electrons.
Get Tom's Hardware's best news and in-depth reviews, straight to your inbox.
But electrons are also free particles – they fight against being ordered this way. In usual conductors, such as the copper wiring that delivers energy to our homes (and is in-built, alongside gold, in our CPUs, irrespective of where in our hierarchy they stand), the electrons can’t zip without losses.
Like files of disorderly, starter-level skaters, some electrons bump against each other, others fall away into holes; it’s a mess. This electronic mess leads to energy leakage and losses; and temperature being simply a measure of how excited subatomic particles are (those skater kids we mentioned), this results in excess work lost as heat. Essentially, electronics heat up because they resist.
Within superconductors, however, there’s no resistance. At that threshold temperature, electrons (our unruly skaters) pair up into Cooper pairs. Bound together at this low temperature (which essentially forces all particles to move slower), electrons can now travel alongside a useful, pre-determined path without being distracted (into wasted energy). As long as they maintain their overall energy levels below the Fermi energy band, they can pass unimpeded, flawlessly delivering electrical energy between point A and point B. Known as BCS theory, owing to its authors Bardeen, Cooper and Schrieffer, the first description of this quantum explanation for superconductivity was published in 1957.
Unfortunately, there’s little that can be done with mercury: it’s not the ideal substance to make working superconductors with. Any substance we want for the purpose of the ideal, dreamt future of superconductors needs to play nicely with our existing techniques and materials. We won’t abandon silicon from our chips just because some strange, expensive mixture of mercury and another material can also be painfully worked into switches and transistors; the costs in doing that would be incalculable.
No; whatever material we come up with must “play ball” with our existing fabrication technology (and global infrastructure) before it revolutionizes the world. We must keep looking.
The Meissner Effect
In 1933, Walter Meissner and Robert Ochsenfeld discovered that superconductors not only facilitated lossless energy conduction, but they also resisted outside magnetic fields. In fact, superconductors “expel” any nearby magnetic fields: the magnetic conduction lines are forced to diverge from the superconducting metal. This repulsion effect came to be known as the Meissner-Ochsenfeld effect (now more generally as the Meissner effect).
Moving back to Maglev trains for a moment, the Transrapid bit refers to the German-made architecture it’s built upon, and it has used both magnetic attraction and magnetic repulsion forces to allow the train to levitate. Since no surfaces are in contact, attrition is reduced to that of air, which allows Maglev trains to be safer and faster than their non-superconducting counterparts. The train’s usage of electrodynamic suspension (EDS) takes advantage of the Meissner Effect’s ability to negate external magnetic fields and to keep it in a position of perfect balance relative to the magnets installed along the “Magway” tramline.
Type I Superconductor
Type I superconductors (the only one we currently know about is tantalum silicide) simply cease to be superconductive as their environment stresses them enough. The material in the superconductive state bursts out of it through a first-order energy transition (reversely equivalent to the same energy transition that led it to become superconductive in the first place), and we’re back to our electrons being high-hormone, disorderly skater kids. This catastrophic loss of function absent the ideal working conditions is what differentiates Type I from Type II superconductors.
For superconductors to be superconductors, they have to present both the ability for their electrons to form Cooper pairs below a certain threshold temperature, as well as a Meissner-effect-induced ability to repulse magnetic fields. But superconduction being essentially a specific form of matter (that threshold state we referred to), it’s common that this state breaks down and ceases to superconduct. The way in which this breakdown happens can take any of two forms, which has led to a division of superconductors into Type I and Type II Superconductors.
Type II Superconductors
Unlike Type I superconductors, which lose their superconductive state of matter in a party-ending burst, Type II superconductors find a way to keep it going even under bouncer supervision. This happens because of how the materials that compose the superconductive material differ between each other.
Because the fabrication process isn’t perfect, particles of material that can transit into the superconducting state of matter are mixed in with other particles that can’t. So when the conditions are just right, parts of the compound will exhibit the superconductive behavior (where electrons travel along as Cooper pairs below the Fermi energy band), while other parts of the compound will exist where electrons are still free to mess things up along their ordered path.
These existential differences create what are essentially gaps in the superconductor compound’s ability to repel magnetic fields. Instead of the fields completely evading the compound, because there are bits of ordinary, non-superconductive material, certain magnetic strings can penetrate the bulk of the material, creating what are known as magnetic field vortices. These vortices lead to an ability called flux-pinning, where levitating materials are pinned in place by the penetration of external magnetic fields.
But what these vortices also do is they put a clock on how the material loses its superconductive state of matter. In the case of Type-II superconductors, the tensions between the superconductive and ordinary state lead to an increase in the vortices’ area; and as the flux-pinning vortices increase in radius and more of the material is penetrated by outside magnetic fields, more of it loses the ability to superconduct. While Type-I superconductors go out with a bang, Type-II superconductors cease working with a whimper.
What's the Outlook for Superconductors?
It’s a fact that our theoretical knowledge of superconduction is incomplete. While we have successfully used YBCO as a high-temperature, high-pressure superconductor, for instance, it’s also true that there’s currently no leading scientific explanation as to why it is that it becomes one.
It’s like watching any complex machine work as it starts, sputters, or spins: we don’t understand how it works, but it’s wondrous that it does. And we’ll be damned if we don’t make it useful.
It’s important to note that superconductors operate at the quantum level – a superconducting material exists when the conditions are there for certain quantum effects to naturally emerge. Condensed-matter physics (the scientific area that concerns itself with supercondcutors) takes into account both chemistry and quantum mechanics (which is simply chemistry at the level of the infinitely small) to study interactions between materials. It’s a complex, rapidly-changing field where breakthroughs are claimed almost weekly.
Zero electrical loss and permanent magnetic fields are entangled within superconductors, and that is big – trillion-dollar market big. Unfortunately, that also means that superconductor research is rife with time and funding sinkholes as well as false gods – retractions and fabricated data are a well-known, high-impact plague within academia, and are hardly limited to this field.
In summer 2023, a Korean paper claiming to have discovered the formula for “The First Room Temperature Superconductor” took the scientific community through an Internet-flowing hype-wave. It’s understandable: we know how important such a material would be. But it’s been a curious case around LK-99, and time will tell if it deserves more than these sentences in the book about superconductors.
But perhaps that’s to be expected of any area of research which “promises” Nobel prizes for the most significant breakthroughs: humans are dreamers that sometimes exaggerate their achievements. And scientists are humans too, and we should avoid letting a few tar the image of every other.
While all the caveats above have to be taken into account, there has been decisive research onto superconduction: this isn’t vaporware. As we mentioned, the Shanghai Transrapid already functions on the basis of superconducting levitation. Particle accelerators such as the Large Hadron Collider (LHC) also employ superconductors, as do MRI machines and certain superconducting cables.
We may not fully understand how superconductors work yet, but perhaps that’s a moot point. Humanity has a habit of putting things to work to our advantage (and detriment) without fully comprehending them. Usefulness doesn’t require complete understanding; otherwise, progress would be grid-locked in amber.
Researchers have a good-enough idea of what to look for on the road to useful room-temperature, ambient-pressure superconduction. But being an explorer is difficult; mapping your way as you go is also considered a high-performance sport, after all.
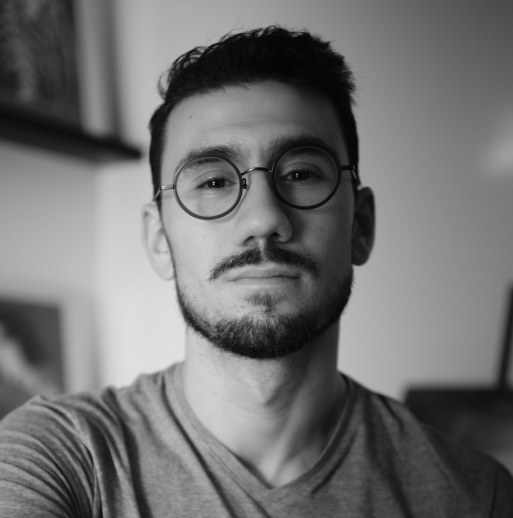
Francisco Pires is a freelance news writer for Tom's Hardware with a soft side for quantum computing.
-
helper800
4.2 kelvin is not 4.2 C. 4.2 kelvin = -268.95 C.Admin said:Onnes was studying the electrical properties of mercury (the same substance that expands and contracts within thermometers) when he found that mercury’s electrical resistance disappeared at temperatures below 4.2 Kelvin (4.2 degrees Celsius).
This is a good generalized article about superconductors and its implications to the tech industry, and even just electrical power distribution in general. -
Geef There is also extra rare 'Super Duper Conductor.' His last name is Higgs and he has Charm along with the ability to conduct a train and an orchestra at the same time! 😜Reply